NAMs can be divided into three broad categories, in chemico, in vitro, and in silico, described in detail below:
In chemico (chemical analysis)
This is generally the simplest NAM technique, in which the chemical reactivity of a substance is measured at a molecular level. These experiments, called in chemico assays, do not require live organisms or even entire cells. Instead, scientists combine test substances with solutions of biological molecules such as peptides, proteins, or DNA to explore what chemical reactions occur. The goal is often to determine whether (or at what concentration or combination) certain chemicals may be toxic. In drug development, in chemico assays are used to gauge a drug’s potential for harm or side effects and how it might interact with other drugs. For nondrug consumer products such as cleaning solutions or cosmetics, in chemico assays are used to check whether the substance might irritate the skin or respiratory system.
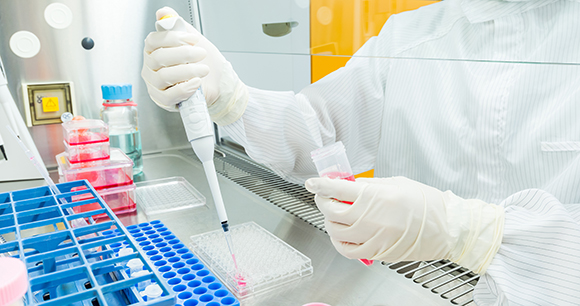
For example, the hemoglobin denaturation (HD) test and the Ocular Irritection test are in chemico alternatives to the Draize rabbit test. The Draize rabbit test was developed in 1944 to test cosmetic products for toxicity and potential to irritate or damage the eye. It involves applying chemicals to the eye or skin of a restrained, awake rabbit, and then scoring the damage to the eye over time—a procedure that often subjects the rabbit to severe pain and discomfort.
Multiple mechanisms are involved in causing eye irritation, but much of it can be explained by the denaturation (breakdown) of proteins and plasma membranes in ocular cells. HD and Ocular Irritection tests combine proteins with solutions of test substances to measure the rate of protein denaturation at different concentrations of the test substance—thus providing a proxy for ocular irritation while avoiding the use of animals. If you're looking for a NAM to replace an animal test or experiment in your lab, consider running an ALTBIB Search, or visit our NAM Resources for Scientists.
In vitro (experimentation on cells)
In vitro (“within the glass,” as opposed to in vivo, “within the living”) refers to experimentation on cells outside of living organisms. Historically, in vitro studies involved growing a single layer of cells on a two-dimensional (2D) plane, such as a petri dish, and then observing them under a microscope to gauge how they react to various substances. As with in chemico testing, the advantage of 2D cell culture experiments is that they are inexpensive and allow for a high degree of control and rapid output. However, this simplicity is also a disadvantage: The isolated and static environment of the cells is not representative of the complex, dynamic, and interactive environment of cells in the body, thus limiting the real-world applicability of 2D cell culture in vitro studies.
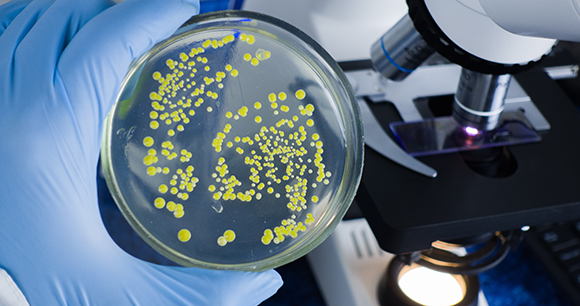
Although 2D cell culture experiments are still useful for answering certain questions, over the past 20–30 years there have been many exciting advancements in other in vitro approaches. Cells can now be grown in three-dimensional (3D) microenvironments that are dynamic and complex, mimicking real organs. These “microphysiological systems” (MPS)—involving “spheroid,” “organoid,” and “organ-on-a-chip” technologies—are completely revolutionizing the way human health research can be conducted.
Spheroids and organoids
Spheroids and organoids are similar, and often the terms are used interchangeably, although there are some technical differences. Both are microscopic, 3D structures composed of multiple cells, but spheroids are less complex than organoids. Spheroids are essentially clusters of cells that stick to one another. Scientists use tumor spheroids (clusters of tumor cells) to better understand the microenvironment of tumors and how effective chemotherapy and other drugs are at treating tumors.
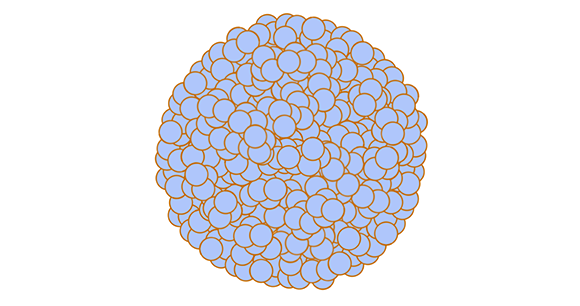
Organoids are miniature 3D versions of organs or tissues that, unlike spheroids, are grown from stem cells. Stem cells are able to follow their own genetic instructions to divide, differentiate, and self-organize to form all the different organs and tissues in the body. This makes organoids more complex than spheroids, as spheroids cannot self-assemble or regenerate. Organoids closely mimic the structure and function of organs and tissues in the body, so they are useful for researchers who want to learn more about the parent organ an organoid represents, such as the liver or the brain.
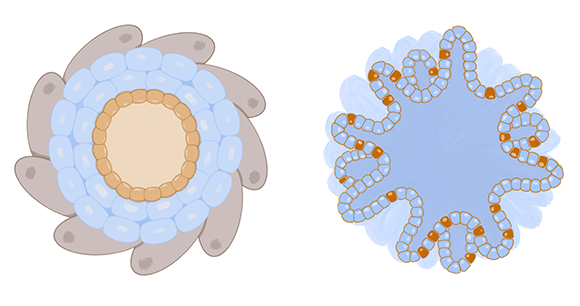
Organoids and spheroids are “closed” systems, in that they lack connections to other organs, and there is no blood flow to transfer oxygen, nutrients, immune cells, waste, and other molecules. For example, a lung organoid can’t expand and contract like a real lung or interact with the flow of oxygen and other aerosols. However, there are even more complex systems called organs-on-a-chip (described below) that do allow for molecular flow, direct interactions between different types of tissues, and a dynamic environment.
Organs-on-a-chip (OOCs; aka “organ chips”)
Organ chips, the most complex in vitro technology, are small devices made of clear plastic, typically the size of a USB memory stick. The chips contain tiny hollow channels, lined with living human cells, that can simulate things like fluid flow, oxygen gradients, and circulating immune cells. In contrast to organoids, organ chips allow for the transfer of nutrients, waste, and drugs back and forth across tissues, mimicking how organs function in real life (e.g., blood flowing through veins; food and fluids moving through the digestive track).
Furthermore, multiple organ chips can be fluidically linked to form multiorgan interactive systems, allowing cell-cell and organ-organ communication. Thus, organ chips present more complex, naturalistic, and dynamic environments that enable cells to behave as they would in a living organism. “They are essentially living, three-dimensional cross-sections of major functional units of whole living organs,” said Dr. Donald Ingber, founding director of Harvard’s Wyss Institute for Biologically Inspired Engineering, which launched this technology in 2009.
The lung-on-a-chip, for example, mimics a breathing human lung, with human lung cells in contact with human capillary blood vessel cells. “And then [by adding simulated breathing motions] the whole thing stretches and relaxes just like our lung does when we breathe,” said Ingber. Researchers have created various other organs chips, including kidney, liver, and heart chips. Ingber and his colleagues are currently working to build a body-on-a-chip, with 10 different organ chips linked together.
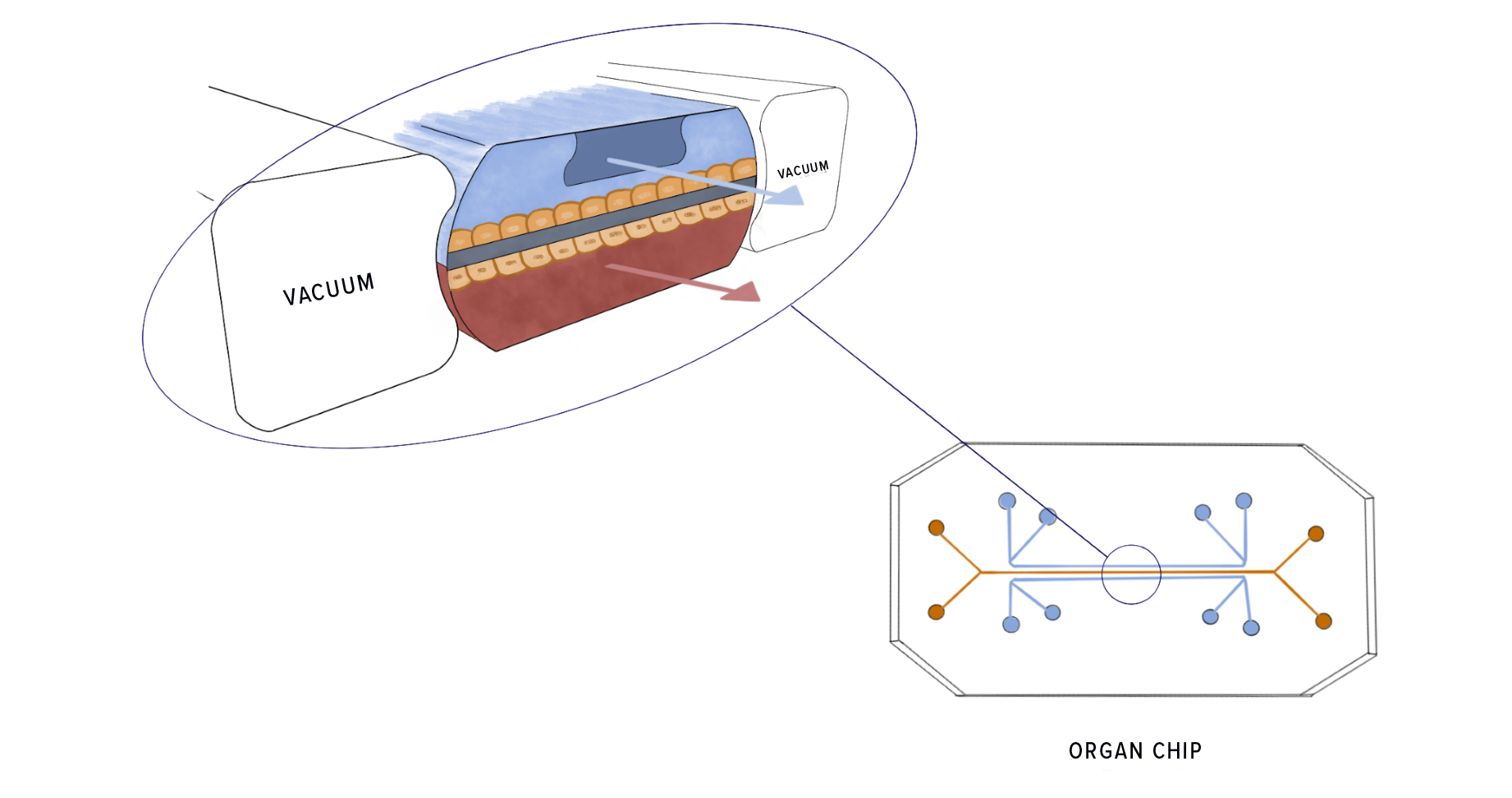
View a video of a lung-on-a-chip in action
Organ chips can be used for a wide variety of purposes: They can be used to test for drug efficacy and toxicity, to explore new biological pathways and disease mechanisms, to investigate the effects of space travel on organs and tissues, or even for wild animal conservation efforts. They can also be used in “personalized medicine,” where they are constructed using a person’s own cells, making them highly specific to that particular individual.
Virtually any organ can be mimicked using this technology, and studying biological effects via organ chips allows for greater human relevance than in vivo studies without causing animals to suffer and/or be sacrificed. For example, in one study, liver chips were used to evaluate 27 drugs known to be either safe or toxic to the human liver. All of the drugs had been characterized as “safe” based on data from animal testing, even though some of the drugs were ultimately found to be toxic to humans in clinical trials. The liver chips correctly identified 87% of the toxic drugs (and did not label any of the safe drugs as toxic).
In silico (computational modeling)
In silico (“in silicon”) refers to experiments performed by a computer via techniques such as mathematical modeling and simulation, machine learning, and artificial intelligence. Using data from previous in vivo, in vitro, or in chemico experiments, computers create simulations of different disease or drug outcomes and test out different factors to see how those factors affect outcomes. The benefit of in silico modeling is that computers can simulate a completely controlled environment, with every detail chosen by the experimenter, and virtually unlimited frameworks can be tested. Rather than investigating cells or systems in isolation, a computer can model an organism in its entirety, exposed to any and every possible environment or substance.
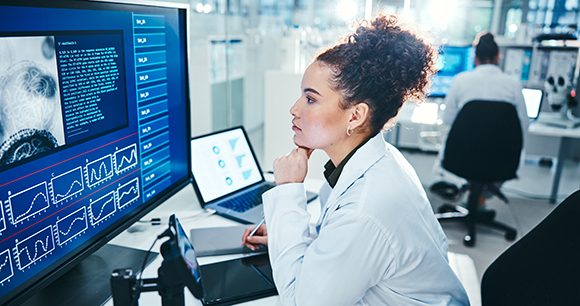
In silico techniques can save time, money, and human and animal lives by scanning through infinite iterations of combinations and possibilities in a way that and is often more accurate and reliable than animal testing (which would require the use of dozens of animals) and could never be feasible through human trial-and-error methods. In the field of drug development, for example, computer software can parse through enormous datasets in a matter of moments and predict possible therapies or toxic properties based on the data provided. It can scan a virtual library of target molecules and then generate a “binding score” for each molecule that can in turn be matched to possible medication. It can analyze the 3D structure of a target molecule and create from scratch a possible treatment molecule based on the structure of a target molecule.
In short, in silico methodologies are nearly limitless in their potential when used in combination with in vivo, in vitro, or in chemico data; however, their outputs are only as reliable as the data that are inputted, so in silico technologies can only be successful if there is access to complete, high-quality data, and it is difficult to validate in silico forecasts (i.e., confirm the results are accurate, reliable, and precise)—although scientists are already working to harness AI to provide such validation.